Technical Note: Illuminated NMR in Chemical Analysis
Dr. Chingyu Chou
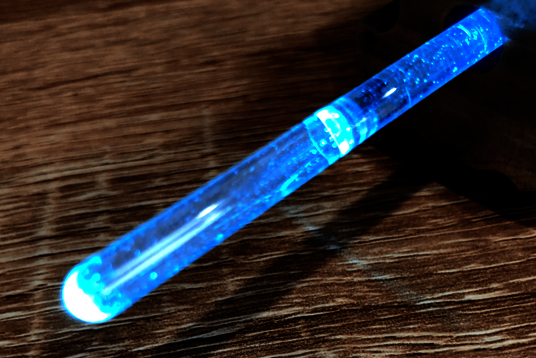
Illuminated Nuclear Magnetic Resonance (NMR) introduces a realm of applications that blend NMR spectroscopy with controlled light irradiation, opening new avenues for exploring chemical reactions and dynamic processes. Within this approach, a significant focus emerges on the captivating phenomenon of Photo-CIDNP (Chemically Induced Dynamic Nuclear Polarization), presenting applications that span diverse scientific domains [1-5]:
- Deciphering Radical Reactions: At the heart of illuminated NMR lies its role in unraveling Photo-CIDNP mechanisms. This phenomenon enhances NMR signals through polarization transfer from photoexcited radical pairs to surrounding nuclei. This understanding provides unprecedented insights into radical reactions, revealing kinetics and spatial arrangements within molecules.
- Dynamic Molecular Insights: The technique's signal enhancement capability sheds light on dynamic molecular processes, such as photoisomerization. Illuminated NMR captures transient states and pathways of isomeric transformations. It also reveals structural changes in photodegradation processes, unveiling molecular stability under light.
- Unveiling Hidden Pathways: Illuminated NMR uncovers "dark" reactions initiated by photochemistry. This impact resonates in fields like organic synthesis, where hidden chemical pathways hold transformative potential.
- Biomolecular Exploration: Illuminated NMR advances biomolecular research by dissecting light-biomolecule interactions. It is vital for retinal proteins, elucidating their conformational changes upon light absorption and their roles in vision and beyond.
In short, illuminated NMR, anchored by Photo-CIDNP, offers a transformative perspective on light-matter interactions. With evolving technology, it redefines molecular dynamics, radical reactivity, and photochemistry's intricacies. By revealing the molecular world under light's influence, illuminated NMR promises significant breakthroughs across disciplines.
The augmentation of sensitivity and the facilitation of chemical reactions hinge on the efficiency of the sample irradiation mechanism. Consequently, the selection of appropriate light sources emerges as a critical consideration.
Correlation Between Irradiation Parameters and Chemical Reaction:
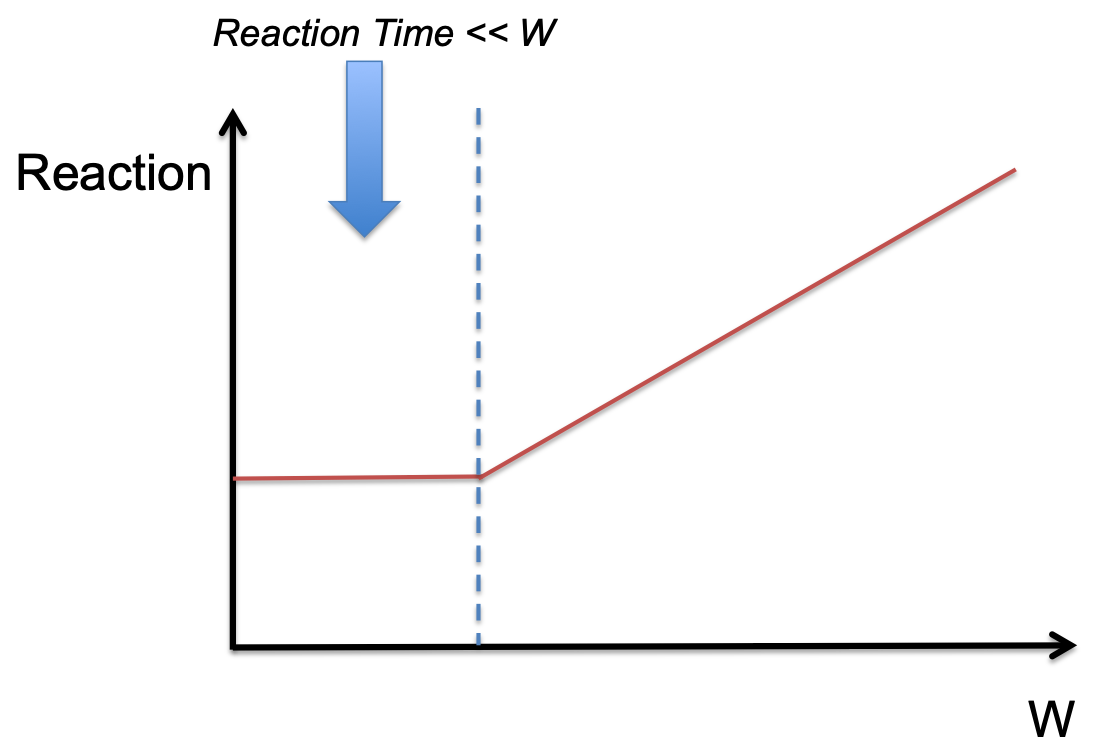
The relationship between total energy and chemical reaction kinetics is a fundamental parameter of illuminated NMR experiments. In this context, the total energy is the product of the irradiate power (P) and the irradiated pulse width (W), i.e., Q = P × W. Understanding the correlation between Q and the chemical reaction rate offers valuable insights into controlling reaction pathways and rates through light irradiation [6].
The total energy, represented by Q, exhibits a pronounced correlation with the total amount of reaction. Manipulating the total energy enables researchers to modulate the kinetics. To decelerate the reaction, reducing the total energy becomes a viable strategy.
In cases where the total energy Q remains constant, a key parameter to manipulate is the irradiate pulse width (W). While maintaining Q as a constant, researchers can modulate the chemical reaction rate by varying W. This approach functions when the timescale of the chemical reaction is significantly shorter than the irradiate pulse width W.
When examining the chemical reaction profile as a function of pulse width, as the reaction timescales are much shorter than W, the chemical reaction rate remains constant since the reaction completes before the full irradiation pulse is delivered. However, as the chemical reaction time approaches or exceeds the irradiate pulse width (W), the reaction rate is correlated with W.
This behavior can attribute to the fact that when the chemical reaction time is comparable to or exceeds W, the reaction has more exposure to the energy delivered by the light pulse. Consequently, longer pulse widths lead to a higher probability of energy absorption by the reacting molecules, resulting in an increased reaction rate.
In summary, the correlation between total energy (Q) and chemical reaction kinetics underscores the significance of irradiation parameters in illuminated NMR experiments. By manipulating the pulse width while keeping the input energy constant, researchers gain a versatile tool to control and tailor reaction rates. Understanding the intricate relationship between Q, pulse width, and reaction kinetics paves the way for precise modulation of photochemical processes in various applications.
Light Source Selection: Laser and LED
Light sources constitute a fundamental aspect of illuminated NMR setups, and two common choices are lasers and Light-Emitting Diodes (LEDs).
- Lasers possess several advantages that make them valuable candidates for illuminated NMR studies:
- High irradiance: Lasers deliver high-intensity, in the scale of Watt.
- Monochromaticity: The monochromatic nature of laser light provides a narrow band of wavelengths for photon interaction.
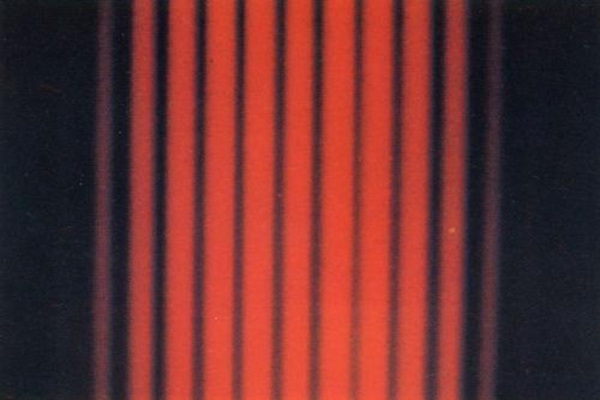
-
However, lasers also come with notable disadvantages:
- Complex Optical Power Control: Achieving consistent optical power control with lasers can be challenging. Laser modules often require integration with photodiodes and closed-loop feedback systems. This complexity demands additional space and positioning outside the NMR magnet, connected via lengthy fiber optics to deliver light into the magnet.
- Coherence-Induced Inhomogeneity: The coherence of laser light can introduce interference patterns within the sample, leading to uneven irradiation. This coherence-induced phenomenon may compromise the reliability and reproducibility of experimental outcomes.
- Limited Pulse Width Control: High-power lasers might struggle to achieve short pulse widths, limiting their suitability for experiments demanding rapid and precise photochemical reactions.
- limited wavelength selection
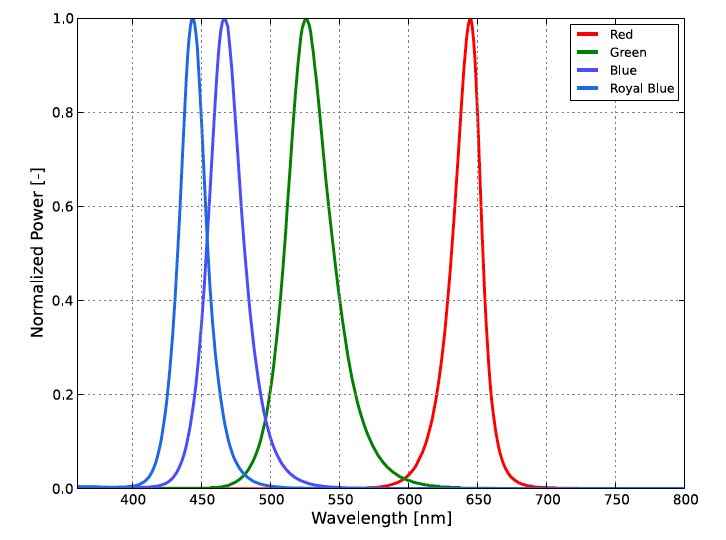
-
LEDs offer a different set of advantages that make them well-suited for illuminated NMR applications:
- Broad Spectrum Emission: LEDs emit polychromatic light covering a range of wavelengths. This characteristic is advantageous for investigating diverse photochemical reactions and processes simultaneously.
- Stable power control: LED setups are relatively simple to configure the power control compared to lasers. It has a positive correlation to the currents.
- *Lab safety: LED has lower lab safety requirements than lasers.
-
Nonetheless, LEDs also have some drawbacks:
- Wavelength Control: While LEDs provide a wide range of wavelength, it also has less concentration of irradiated wavelength as a reaction needs precise wavelength-irradiation.
- Lower Irradiance: LEDs have a micro-W to W power range. Compared to lasers, LEDs, in general, are less powerful.
Lasers offer the advantage of delivering high-intensity, monochromatic light, allowing precise targeting of specific wavelengths. They are especially advantageous for studies requiring fine-tuned photon energies. However, these advantages can become disadvantages of lasers. The inherent properties of lasers can lead to interference patterns and uneven irradiation, necessitating additional measures to mitigate this light interference when lasers as the light source.
LEDs provide a versatile alternative. Emitting polychromatic light, LEDs cover a wide range of wavelengths. The broad emission spectrum does not have interference patterns and can reach homogenous irradiation without additional effort. However, when an interaction requires a specific wavelength to irradiate, this becomes an unavoidable shortage.
In photo-CIDNP applications, the magnetic field-dependent factor plays a pivotal role [3]. Sensitivity enhancement in photo-CIDNP is a function of magnetic field strength. The ideal setup for achieving high sensitivity and high-resolution photo-CIDNP entails a high-field field-cycling NMR system integrated with a sample illumination mechanism.
Consider the scenario of a sample shuttling system within a high-field NMR spectrometer. Here, the light source module must optimally occupy minimal space while delivering homogenous sample irradiation. Exceptionally, LEDs turn into a superior choice for high-field field-cycling NMR setups. Modern LEDs can generate substantial power outputs, rivaling those of lasers. It eliminates the traditional power disparity between lasers and LEDs. Furthermore, by positioning LEDs closer to the sample, the power loss associated with optical fiber transmission, inherent in laser setups, is circumvented. This approach minimizes the power gap between LED and laser sources, effectively equalizing their end optical powers.
As LEDs are situated proximally to the sample for efficient irradiation, the requirement for LEDs to possess lower optical power than lasers becomes unnecessary. The practical advantage of LEDs, coupled with their comparable power levels and simplified setup, positions them as a favorable light source for high-field field-cycling NMR systems in photo-CIDNP experiments.
In conclusion, addressing issues of light homogeneity and optimizing sensitivity are crucial considerations when selecting light sources for photo-CIDNP applications. The distinctive advantages of LEDs, including power output and proximity to the sample, make them an attractive choice for high-field field-cycling NMR setups, bridging the power gap with lasers and offering a practical solution for achieving both sensitivity and precision in photo-CIDNP experiments.
Related Instruments
References
- Fedin, M.V., E.G. Bagryanskaya, and P.A. Purtov, Theoretical and experimental studies of chemically induced dynamic nuclear polarization kinetics in recombination of radical pairs by the method of switched external magnetic field. II. 13C CIDNP of micellized radical pairs. The Journal of Chemical Physics, 1999. 111(12): p. 5491-5502.
- Kuhn, L.T., Photo-CIDNP NMR Spectroscopy of Amino Acids and Proteins. 2013. 338: p. 229-300.
- Magin, I.M., et al., Low field photo-CIDNP in the intramolecular electron transfer of naproxen–pyrrolidine dyads. Physical Chemistry Chemical Physics, 2016. 18(2): p. 901-907.
- Seegerer, A., P. Nitschke, and R.M. Gschwind, Combined In Situ Illumination-NMR-UV/Vis Spectroscopy: A New Mechanistic Tool in Photochemistry. Angewandte Chemie International Edition, 2018. 57(25): p. 7493-7497.
- Ji, Y., et al., LED‐Illuminated NMR Spectroscopy: A Practical Tool for Mechanistic Studies of Photochemical Reactions. ChemPhotoChem, 2019. 3(10): p. 984-992.
- Kaptein, R. (1982). Photo-CIDNP Studies of Proteins. In: Berliner, L.J., Reuben, J. (eds) Biological Magnetic Resonance. Springer, Boston, MA. https://doi.org/10.1007/978-1-4615-6540-6_3