A little personal reminiscence of the early days of field-cycling NMR relaxometry
Prof. Rainer Kimmch / University of Ulm, Germany
When people who are interested in molecular dynamics start investigations based on field-cycling NMR techniques they can nowadays employ mature, welldesigned and versatile systems that are commercially available. They can just focus on the samples of their research interest and rely on the hard- and soft-ware offered for such studies. Sixty years back, in the sixties of the last century, tedious and time-consuming preparations often for years were required before any relaxometry data point could be recorded. Anyway, at that time it was clear that it would be most promising to exploit the field/frequency dependence of relaxation times or rates for the characterization of molecular dynamics: There was high motivation to take efforts to try relaxation dispersion experiments.
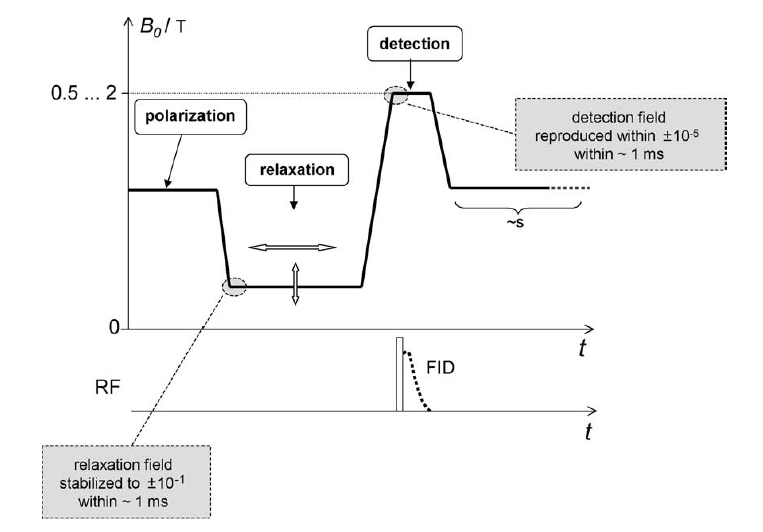
The way to reach this goal was to develop and build instruments from the scratch on one’s own. This includes the entire NMR RF system since there were no commercial suppliers offering more than rather weak RF amplifiers and iron electro-magnets at most. In the lab at the University of Stuttgart in Germany where I wrote my PhD thesis at that time, Friedrich Noack had already practiced NMR relaxation dispersion experiments using a multi-channel system operating at 15 discrete frequencies logarithmically distributed between 50 kHz and 160 MHz. Actually, the essentially only component common to all these channels was a steady-state electro-magnet that could be adjusted to the resonance field currently needed. Apart from that, different transmitters, receivers, probeheads and – quite crucially – different sample sizes from a tenth up to 50 ml had to be employed depending on the frequency. One could say that this was a kind of “agglomerate” of 15 separate NMR devices. Not to mention how laborious the handling of such a system was, and how difficult it was to record transient NMR signals visually on the fly with non-storage oscilloscopes in lab rooms darkened just for this purpose. For better or worse, the human eye had to act as analog-to-digital converter!
Ironically, I heard later from a Japanese colleague who wanted to avoid such hardship. He took his samples and travelled from NMR lab to NMR lab hoping to find spectrometers tuned to frequencies in a wide range. Actually, at that time variable electro-magnets were in use, and since all NMR systems were home-made, there was a substantial variation of the resonance frequencies employed. So, the Japanese colleague was not entirely unsuccessful with his strategy.
In the sixties of the last century, another rudimentary effort to measure relaxation dispersion was undertaken in the Stuttgart NMR lab: A simple sample shuttle device. Since spin-lattice relaxation in the systems of interest at that time, namely crystals of pure water ice, is extremely slow, it was adequate to shift the sample manually between two electro-magnets back and forth, one magnet on resonance and the other one adjusted to a variable field. It is needless to say that the poor field homogeneity and reproducibility did not permit the record of any highly resolved NMR spectra. No doubt, all these attempts were cumbersome, time consuming and rather inaccurate.
It thus became soon clear that electronically operated field variation devices would be desirable, either by using switchable power supplies for the magnet or by employing electronically controlled sample shuttle systems linking different fields in transfer times as short as possible. In my PhD thesis, I was interested in molecular dynamics of protein solutions which I wanted to examine with the aid of proton spin-lattice relaxation dispersion. The first part of my job was therefore to design and build an electronic field-cycling device. However, there was no literature available how to do that. So I had to come up with my own solution. At the same time, several groups around the world independently worked on the same problem and ended up with quite different technical designs. This in particular refers to Alfred Redfield’s group in Yorktown Heights and Jacek Hennel’s department in Krakow.
In my case, I had a switchable 1 kW power supply on hand. The bandwidth was somewhat more than 1 kHz. Since this is not enough for the generation of reasonably strong and homogeneous detection fields in magnet coils, I constructed a field compensation device: It consisted of a large water-cooled air coil operated with a steady-state power supply to produce a homogeneous enough detection field. In the relaxation interval, this field was variably und partially compensated at the sample position with the aid of a small, concentric and low-inductance coil connected to the mentioned switchable power supply. Thus, short switching time (milliseconds) could be achieved in combination with a relatively high and homogeneous detection field (up to 1 MHz proton resonance), while the homogeneity of the field in the relaxation interval was uncritical.
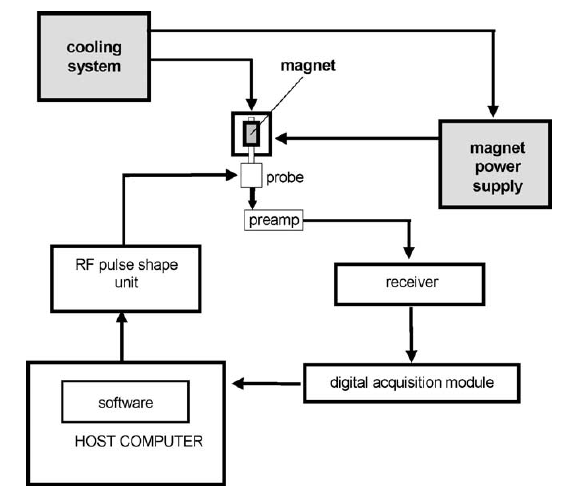
This was the state in the Stuttgart lab in the late sixties. The main effort in subsequent years was to increase the detection field in order to improve the detection sensitivity. At the end of the day, this is a matter of how to cool the magnet sufficiently in view of the huge currents fed in. In my lab at the University of Ulm, we were using a liquid-nitrogen magnet coil for a couple of years. The principle was analogous to the design Seymour Koenig had established in Yorktown Heights. A further step towards higher detection fields was achieved with the aid of superconducting coils. 1.5 Tesla could be achieved, but the consumption of liquid helium was enormous. Finally our budget was made easier in this respect, since a young student accidentally operated the magnet without refilling liquid helium: The magnet coil was consequently damaged beyond repair. With a later version of a superconducting magnet coil we even achieved 3 Tesla, but the helium consumption problem remained. So, liquid-cooled, room temperature, one-to-two Tesla magnets of optimized coil architecture turned out to be the best compromise. This is now the standard both with home-built and commercial field-cycling relaxometers.
One small episode, which is hard to imagine in times of globalization, should be mentioned in passing: In the seventies, we used a power supply unit from a small French company for our field-cycling magnets. One day, we had to send the device back to the company for repair, and that's where the problem started. Mind you, that was before the establishment of the free market in the EU. So we sent the power supply with a German trucking company to the customs at the German-French border. There a French forwarding agency took over the shipment and was supposed to transport it to the manufacturing company after release by customs. But what arrived there was a pile of irreparable scrap. Somewhere along the way from Ulm to the French manufacturer, someone had dropped the expensive device from a truck or from a ramp. But nobody wanted it to be, and a months-long legal battle ensued until we received a small financial compensation from the insurance of one of the involved companies.
With a detection-field homogeneity and reproducibility of 10-5 at best, there is no chance to acquire highly resolved NMR spectra with electronically switched fieldcycling relaxometers. This is rather the realm of sample shuttle devices that have been developed by Alfred Redfield and other groups to a top standard in the same decades-long period. Interestingly, homogeneous, i.e. perfectly shimmed detection fields (in the center of superconducting magnets) are again combined with relatively inhomogeneous, but sufficiently low relaxation flux densites (in the fringe field of the magnets). In conclusion, we now have mature commercial solutions available for both the one and the other technology (Field-cycling NMR technique), and users can concentrate on their real research issues.
Figure Reference
R. Kimmich, E. Anoardo / Progress in Nuclear Magnetic Resonance Spectroscopy 44 (2004) 257–320